- 1Department of Earth and Environmental Sciences, University of Kentucky, Lexington, KY, United States
- 2Department of Earth and Environmental Systems, Indiana State University, Terre Haute, IN, United States
Jackson Lake supplies valuable cultural and provisioning ecosystem services to the Upper Snake River watershed in Wyoming and Idaho (western USA). Construction of Jackson Lake Dam in the early 20th century raised lake level by ∼12 m, generating an important water resource supporting agriculture and ranching, as well as tourism associated with Grand Teton National Park. Outlet engineering drastically altered Jackson Lake’s surface area, morphology, and relationship with the inflowing Snake River, yet the consequences for nutrient dynamics and algae in the lake are unknown. Here, we report the results of a retrospective environmental assessment completed for Jackson Lake using a paleolimnological approach. Paleoecological (diatoms) and geochemical datasets were developed on a well-dated sediment core and compared with available hydroclimate data from the region, to assess patterns of limnological change. The core spans the termination of the Little Ice Age and extends to the present day (∼1654–2019 CE). Diatom assemblages prior to dam installation are characterized by high relative abundances of plankton that thrive under low nutrient availability, most likely resulting from prolonged seasonal ice cover and perhaps a single, short episode of deep convective mixing. Following dam construction, diatom assemblages shifted to planktic species that favor more nutrient-rich waters. Elemental abundances of sedimentary nitrogen and phosphorous support the interpretation that dam installation resulted in a more mesotrophic state in Jackson Lake after ∼1916 CE. The data are consistent with enhanced nutrient loading associated with dam emplacement, which inundated deltaic wetlands and nearshore vegetation, and perhaps increased water residence times. The results of the study highlight the sensitivity of algal composition and productivity to changes in nutrient status that accompany outlet engineering of natural lakes by humans and have implications for water resource management.
Introduction
Reservoirs provide substantial social, ecosystem and economic benefits, as dams transform rivers or lakes into regulated water resources (e.g., Postel and Carpenter, 1997; Graf, 2001; Lehner et al., 2011; Sawakuchi et al., 2015). Cost-benefit analyses frequently document tradeoffs associated with dam construction vis-à-vis natural regulating, provisioning, supporting, and cultural ecosystem services (e.g., WCD, 2000; Intralawan et al., 2018; Wild et al., 2019). For example, dams built on free-flowing rivers may lead to shifts in aquatic primary production, degrade biodiversity as habitats are lost, or affect fisheries, in addition to altering water and sediment budgets and generating hydropower (e.g., Graf, 2005; McCartney, 2009; Song et al., 2019). In the early 20th century, numerous dam projects were carried out in the western USA by the U.S. Bureau of Reclamation to support the water demands of expanding agricultural and ranching interests (e.g., Graf, 2001). Early dam projects, such as the Minidoka Project in the Upper Snake River watershed, converted several natural lakes into reservoirs through outlet engineering to provide power generation, flooding control and irrigation water (Stene, 1997). From 1906 to 1939, five major dams were completed: Minidoka, Jackson Lake, American Falls, Island Park, and Grassy Lake. Determining the ecological impacts of dam emplacement is a challenge for reservoirs that have existed for more than a century, as baseline limnological monitoring data are often absent or incomplete. In such cases, paleolimnological studies utilizing lake sediment cores open the opportunity for retrospective environmental assessments (sensu Smol, 2019). This study examines the ecological impacts of dam construction on Jackson Lake, one of the largest reservoirs in the Upper Snake River watershed of Wyoming, using fossil diatoms and geochemical signals preserved in lake sediments. Jackson Lake dam is a concrete gravity structure with an earthen dike that was completed in 1916. The resulting reservoir increased lake level by ∼12 m and lake surface area from 27.2 km2 to 40.5 km2, flooding marginal coastlines and wetlands (Figure 1). Dam installation on the southeastern corner of Jackson Lake made the reservoir capable of storing 1.04 × 109 m3 (847,000 acre-feet) of water, the largest in the region (Stene, 1997; Daugherty et al., 1999). In addition to water resources for farming and ranching, Jackson Lake provides numerous other valuable cultural ecosystem services today as a recreational focal point in Grand Teton National Park. Jackson Lake serves as important trout habitat, and angling, camping, and recreational boating are major seasonal tourist attractions (Wyoming Game and Fish Department Fish Stocking Reports, 2021). Grand Teton National Park attracted ∼3.3 million visitors in 2020, which resulted in ∼$754 million of revenue added to the local economy (Thomas and Koontz, 2021).
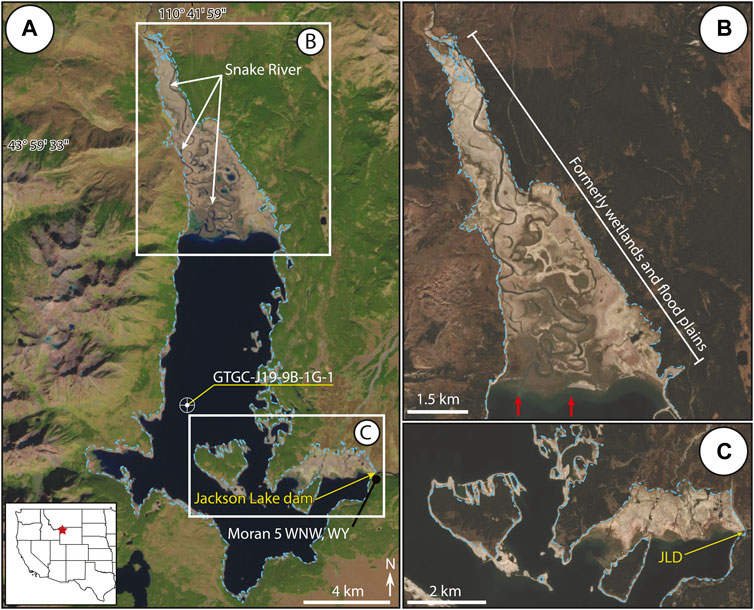
FIGURE 1. Jackson Lake is the largest of the piedmont lakes within Grand Teton National Park. The Snake River enters from the north forming the Snake River Delta. Anthropogenic features include the Jackson Lake Dam (JLD) and the weather station Moran 5 WNW, WY. The location of sediment core GTGC-J19-9B-1G-1 is denoted by the bullseye. (A) Landsat image of Jackson Lake taken 10/4/2021 at the end of the summer drought—this configuration is likely analogous to how the lake would have appeared prior to installation of the JLD. Blue dotted line represents the lake margin when the reservoir is at full capacity. (B) Sentinel image illustrating subaerial exposure of northern Jackson Lake during lowstand. Red arrows indicate pre-dam delta position. (C) Sentinel image displaying the emergence of the southeastern corner of Jackson Lake near the JLD.
Jackson Lake’s ecological responses to changes in climate and hydrology over the past several centuries are largely unknown. According to Hostetler et al. (2021), from 1950 to 2018 CE temperatures warmed in the Snake River headwaters by 1.1°F and in the Upper Snake River by 2.3°F. Snowpack volume and mean annual snow fall have both declined, and the initiation of snowmelt is projected to shift 1–2 months earlier in the future. The timing of snowmelt, more so than rainfall, is important for the recharge of rivers and lakes in Grand Teton National Park. It is projected that summers and winters will continue to warm in the Teton Range, with precipitation projected to increase by 7%–8% by the end of the century (Hostetler et al., 2021). Presently, average annual temperatures in the nearby city of Jackson Hole have warmed, but precipitation (as rain) has remained consistent from 1910 – present (NOAA, 2021a; NOAA, 2021b). Predictions of warmer summers, declines in winter snowpack, a shift to less winter snowfall, and increases in annual rainfall make sustainable management of Jackson Lake increasingly critical, and challenging.
Completion of Jackson Lake Dam expanded the lake’s surface area by ∼30%, which flooded the Snake River delta and marginal wetlands (Stene, 1997; Daugherty et al., 1999). Although water level at Jackson Lake has been monitored daily by the U.S. Bureau of Reclamation since dam completion, limnological datasets that span the pre- and post-dam interval are unavailable. Dam emplacement on other natural lakes has been shown to alter algal productivity, sediment fluxes, nutrient inventories, and water residence times, in addition to increasing lake-level elevations and altering discharge at the outlet (e.g., Baxter, 1977; Finger et al., 2006; Matzinger et al., 2007). Given Jackson Lake’s importance to the region, a retrospective environmental assessment was undertaken using a well-dated sediment core (GTGC-J19-9B-1G-1) to assess changes to algal communities and productivity in this ecosystem from ∼1654 to 2019 CE. Fossil diatom assemblages were used as indicators of nutrient status and water column mixing state. Diatoms are microscopic photosynthetic algae that produce siliceous ornamented cell walls (frustules) consisting of two valves separated by girdle bands (Battarbee et al., 2001a). Diatoms proliferate rapidly, often in direct response to changes in nutrient conditions, hydrochemistry, and lake levels, making their assemblages and abundances useful ecological indicators in many different types of inland waters (e.g., Battarbee et al., 2001b; Smol and Stoermer, 2010; Guerreiro et al., 2018; Kamulali et al., 2022). A companion paper (McGlue et al., 2023) describes the core’s age-depth model, lithostratigraphy, and geochemical data. Taken together, the datasets provide a new, high-resolution natural and anthropogenic history for Jackson Lake that has implications for ecosystem management and conservation.
Study Site
Jackson Lake is the largest piedmont lake in Grand Teton National Park and is situated in the Jackson Hole sedimentary basin (Figure 1). The bedrock geology surrounding the lake ranges from Late Archean metamorphic to Late Quaternary sedimentary and volcanic rocks (Love et al., 1992; Smith et al., 1993). The Teton fault, a major N–S striking normal fault, produces the topography of the Teton Range and forms the western margin of Jackson Lake (Love and Reed, 1968; Byrd et al., 1994; Pierce and Haller, 2011; Zellman et al., 2019; Thigpen et al., 2021). Three major ice advances originating from the Greater Yellowstone Glacial System during the Late Pleistocene sculpted much of the topography present around Jackson Lake (Good and Pierce, 1996; Pierce et al., 2018). Jackson Lake is believed to have formed by the most recent Pleistocene glacial advance (Pierce et al., 2018). Various moraines and drumlins are found along the eastern and southern lake margins (Figure 1). U-shaped valleys are common among the peaks of the Teton Range, and many of these valleys are occupied by under-fit streams that drain into Jackson Lake from the west (Johnson et al., 2022).
Jackson Lake exhibits an elongated N–S axis with a maximum depth of ∼145 m. The deepest area of the lake is found along the western margin adjacent to the Teton Range with shallower depths common along the eastern margin (Figure 1). The lake’s outlet is located on the southeastern margin. Freshwater inflow to Jackson Lake is chiefly from the Snake River on its northern termination, with smaller lateral margin streams contributing from the escarpment and shoaling margins. Estimates for Snake River inflow from a gauge station ∼10 km north of Jackson Lake (USGS gauge station #13010065) indicate instantaneous stream flow between 5.4 and 195.4 m3/s. (1998–2002 CE; Clark et al., 2004). Measured nutrient concentrations from that gauge station showed ammonia ranging from <0.04 to 0.05 mg/L, nitrate from <0.05 to 0.12 mg/L and phosphorous from <0.06 to 0.41 mg/L (1998–2002 CE, Clark et al., 2004). Inflow rates and nutrient loads from the marginal streams are unknown; given minimal development in the region and the bedrock geology, nutrient levels are assumed to be like those in the Snake River. Nutrients could be contributed to the lake through hydrothermal springs, gas seeps, or regional groundwater flow but contributions from these sources have not been characterized (McGreevy and Gordon, 1964; U.S. Geological Survey, 1978).
Jackson Lake is not well-studied from a limnological perspective, though early geodetic maps of western Wyoming confirm that it has been hydrologically open since at least the mid-19th century. Wurtsbaugh (2014) demonstrated that a late summer thermocline exists in Jackson Lake at 12–15 m below the lake surface and the photic zone extended down to 13 m. Daily water level measurements were recorded by the U.S. Bureau of Reclamation from 1910 to 2022 CE, producing a high-resolution lake-level curve since dam emplacement (Figure 2). Lake-level oscillations can be correlated to fluctuations in the regional semi-arid climate (Curtis and Grimes, 2004). The Teton Range intercepts moisture carried by westerly storms; mean annual precipitation and temperature near Jackson Lake are 152 mm and 10°C, respectively (NOAA, 2021a; NOAA, 2021b). Palmer Drought Severity Index (PDSI) calculations suggest that major droughts occurred in the mid-1930s (Dust Bowl), 1950–1960s, the mid-late 1980s, and the early 2000s (Figure 2). Major droughts resulted in lower reservoir levels, which usually align with low regional PDSI (NOAA, 2021b) (Figure 2). Evaporation, reduced runoff, and downstream water needs influence reservoir levels during droughts. Jackson Lake Dam’s gated spillway allows operators to modulate outflow or cut it off completely, sequestering nutrients and increasing water residence times (U.S. Bureau of Reclamation, 1928). During droughts, such as in 2021, the spillway gates were opened, allowing additional reservoir drawdown to supplement water resources downstream (Figure 1).
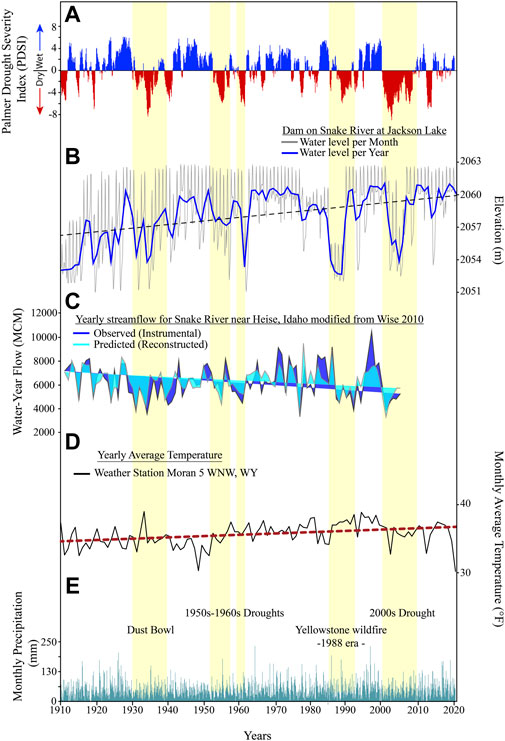
FIGURE 2. Jackson Lake water levels compared against several different hydrologic variables (1910-2022 CE). (A) Palmer Drought Severity Index obtained from the National Integrated Drought Information System (www.drought.gov). Pale-yellow bars, major drought intervals. (B) Monthly average water levels of Jackson Lake. Running average shown in dark blue line. Data was acquired from the U.S. Bureau of Reclamation (https://www.usbr.gov/pn/hydromet/arcread.html). (C) Annual Snake River flow near Heise, Idaho. Observed (dark blue) and predicted from tree-rings (light blue) are plotted for the period of interest (data modified from Wise, 2010). (D) Average annual temperatures (black line) with a long-term trend shown in red (NOAA, 2021a). (E) Monthly average precipitation (NOAA, 2021b).
Methods
Diatom Processing and Microscopy
Age constraints for the diatom sample intervals were determined by the chronological model for core GTGC-J19-9B-1G-1 (hereafter, core 9B) discussed in McGlue et al. (2023) (Figure 3). Core 9B was subsampled for diatoms every 1 cm (n = 57) lengthwise. Individual samples were oxidized with 35% H2O2 and digested at room temperature for 3 weeks to remove organic matter (OM) (Bracht et al., 2008). Following digestion, samples were rinsed with deionized water and placed into individual polyethylene flasks, to which a known concentration of microspheres were added (Battarbee and Kneen, 1982). An aliquot from each flask was placed on a coverslip, dried, and mounted on a glass slide using Naphrax. Examination of diatoms was completed using a Nikon Eclipse LV100N POL light microscope under ×100 magnification. Following the procedure outlined in Battarbee and Kneen (1982), 300 valves were counted per slide. Taxa identification was aided by a scanning electron microscope (SEM) and reference collections at Indiana State University and the Diatoms of North America (DoNA) (Diatoms of North America, 2021) Taxon Identification Guide (Spaulding et al., 2010). Stephanodiscus parvus and Stephanodiscus minutulus identification followed the DoNA taxonomic guide. For SEM analysis, diatom extracts were evaporated onto 10 mm aluminum specimen mounts and attached to stubs using carbon tape. Sample stubs were coated with gold using a Denton Desk V coater (Denton Vacuum, LLC) and imaged using a TESCAN Vega 3 (Tescan USA, Inc.) at 10 kV following the procedure outlined in Stone et al. (2020). Valves per Gram was calculated based on the method of Battarbee and Kneen (1982). A threshold value of 5% of the total assemblage was used to establish the major diatom species in the core; 14 species met this threshold. Species that did not meet the 5% threshold were not evaluated. Relative abundances of the major diatom species were determined, and a constrained incremental sum-of-squares cluster analysis (CONISS) was completed using Tilia version 2.6.1 software (Figure 4).
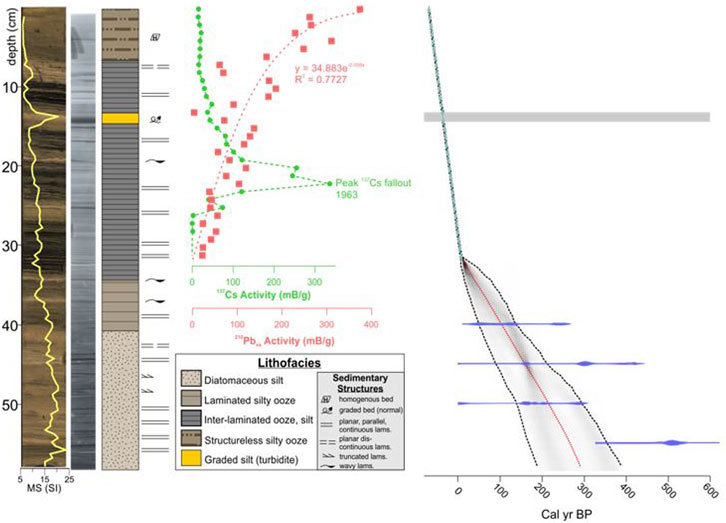
FIGURE 3. High resolution photo, X-radiograph (dense = dark), interpreted lithostratigraphy and Bacon age depth model (14C, 137Cs, 210Pbxs) for core 9B (from McGlue et al., 2023). Grey bar, turbidite excised from the Bacon age model. See text for details.
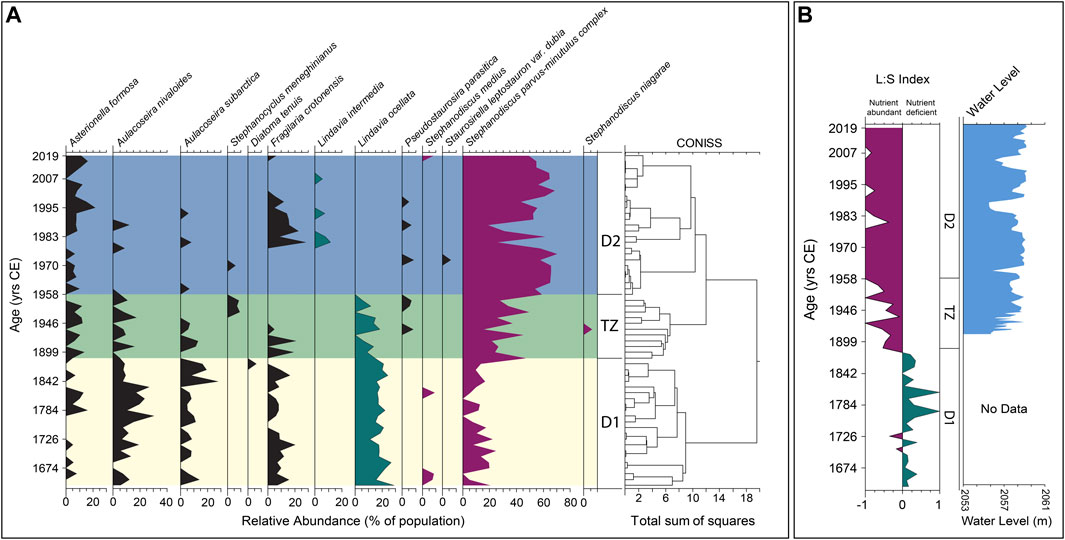
FIGURE 4. (A) Relative abundances of major diatom genera above a 5% threshold of total population. Vertical divisions are constrained by incremental sum-of-squares clustering (CONISS). Diatom Zone 1 (D1; yellow). Transition Zone (TZ; green). Diatom Zone 2 (D2; blue). (B) Lindavia to Stephanodiscus index and Jackson Lake water level data. Positive values indicate prevalence of Lindavia (green), representing relatively nutrient deficient conditions. Negative values represent prevalence of Stephanodiscus (violet), indicating relative nutrient abundance. See text for details.
Lindavia:Stephanodiscus Index (L:S)
By contrasting the relative abundances of Lindavia to Stephanodiscus throughout the core, we inferred changes in lake nutrient status over time. Species belonging to the genus Stephanodiscus are typically associated with eutrophic lakes high in total P (Reavie et al., 1995). Streib et al. (2021) showed that S. minitulus are typically found in water bodies with elevated nutrient concentrations such as in mesotrophic or eutrophic alkaline lakes. In contrast, diatoms belonging to the genus Lindavia are associated with oligotrophic or nutrient-limited aquatic systems but can be found in environments ranging from ultraoligotrophic to eutrophic, with sandy or gravelly substrates (Houk, 2010; Saros and Anderson, 2015; Streib et al., 2021). The Lindavia and Stephanodiscus index (L:S) was calculated according to Streib et al. (2021). Positive index values represent dominance of Lindavia, and high values of L:S are interpreted to reflect lower nutrient status, associated with limited nutrient flux or a stratified water column that limits nutrient cycling into the epilimnion. Negative index values represent dominance of Stephanodiscus and are interpreted to reflect greater nutrient availability in the epilimnion, perhaps linked to more abundant nutrient flux or extensive internal mixing (Figure 4).
X-Ray Fluorescence (XRF) and Sediment Geochemistry
XRF scanning was completed using a Cox Analytical Systems ITRAX XRF core scanner located at the University of Minnesota-Duluth. The core surface was cleaned and scanned using a 15 s dwell time to produce high resolution elemental abundance data for a suite of common elements (P, Si, Ti); data are reported as counts per second (cps). Silicon is a principal element composing diatom frustules, and normalizing Si to Ti provides an indicator of biogenic silica (opal) in lake sediments (e.g., Kylander et al., 2011). Total nitrogen measurements were made using a Thermo Finnigan DELTAplus XP elemental analyzer at the University of Kentucky and are discussed in McGlue et al. (2023).
Results
Diatoms
Diatoms are abundant throughout the core (Figure 4) and are characterized by good valve preservation; evidence of dissolution or fragmentation is absent or rare. Planktic species dominate the record (Supplementary Figure S1). The following species comprise ≥5% of the diatom assemblage: Asterionella formosa, Aulacoseira nivaloides, Aulacoseira subarctica, Stephanocyclus meneghinianus, Diatoma tenuis, Fragilaria crotonensis, Lindavia intermedia, Lindavia ocellata, Pseudostaurosira parasitica, Staurosirella leptostauron var. dubia, Stephanodiscus medius, Stephanodiscus niagarae, Stephanodiscus parvus and Stephanodiscus minutulus. Similarities in occurrences between S. parvus and S. minutulus led to the interpretation of a single complex, hereafter referred to as S. parvus-minutulus. The CONISS analysis (Figure 4) indicates two main clusters separated by a transitional group. Diatom zone 1 (hereafter, D1) occurs from 57 to 36 cm (1654–1888 CE) (Figure 4). The transition zone (hereafter, TZ) occurs from 36 to 25 cm (1888–1958 CE) (Figure 4). Diatom zone 2 (hereafter, D2) extends from 25 cm to the top of the core (1958–2019 CE) (Figure 4).
Diatom Zone 1—1654–1888 CE
Diatom zone 1 exhibits consistent abundances (∼20%, Figure 4) of L. ocellata moving towards the upper zonal boundary, with varied assemblages of S. parvus-minutulus, A. formosa, A. nivaloides, A. subarctica and F. crotonensis. The most representative Lindavia species, L. ocellata, maintains a consistent relative abundance of ∼20%, with rare peaks of 25%–30%, with two larger peaks at 1658 and 1697 CE (Figure 4). Contrasting with Lindavia, abundances of the S. parvus-minutulus complex exhibit a more variable pattern throughout D1, averaging ∼10% with rare peaks up to ∼25% (Figure 4). The largest peak abundance (∼45%) of S. parvus-minutulus occurs at 36 cm (1888 CE) at the boundary with the TZ (Figure 4). The L:S ratio shows variable but dominantly positive values in D1 (Figure 4), with transient negative peaks where Stephanodiscus abundances increase. Asterionella formosa occurs infrequently throughout D1 (Figure 4), with abundances rarely exceeding 6%–7%. Increases in A. formosa generally occur with declines in F. crotonensis (Figure 4). Aulacoseira nivaloides and A. subarctica assemblages occur coevally, with the former generally more abundant. The largest peaks of A. nivaloides occur at 46 cm (1773 CE) and 41 cm (1830 CE), while the largest peaks for A. subarctica occur at 40 cm (1842 CE) (Figure 4). Fragilaria crotonensis is commonly present under ∼10%, with three peaks reaching 15% at 57 cm (1658 CE), 51 cm (1716 CE) and 39 cm (1853 CE) (Figure 4). Diatom concentrations show a variable but increasing trend throughout D1 (Figure 5). Geochemical data are reported for Si:Ti, TN and P (Figure 5). The Si:Ti chemostratigraphy exhibits a variable negative trend throughout D1 with values ranging from ∼0.18 to 0.29 (Figure 5). Total nitrogen concentrations show no clear trend in D1 with mean values ∼0.2 wt. % (Figure 5). High variability in P is evident in D1, with no discernable trends.
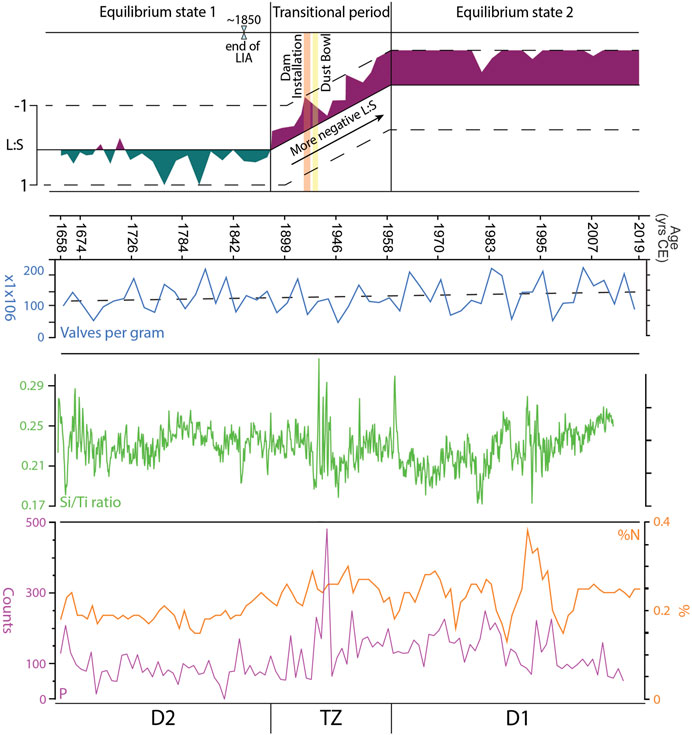
FIGURE 5. Equilibrium state 1 of Jackson Lake occurred during 1685–1888 CE and is characterized by positive L:S values, with transient negative peaks. The Little Ice Age (LIA) is present in equilibrium state 1 from 1685 to its end in 1850 CE. Diatom Zone 2 (D2) occurs during equilibrium state 1. From 1888 to 1958 CE, Jackson Lake was in a state of transition. L:S values during this period are consistently negative but vary in intensity. During this time dam installation was completed (1906–1916 CE) and the Dust Bowl occurred (1930–1939 CE). Equilibrium state 2 occurred between 1958 and 2019 CE where L:S stabilizes at strongly negative values. Diatom Zone 1 (D1) occurs within equilibrium state 2. Valves per gram and Si:Ti data suggest that overall lake productivity has not changed dramatically over the period of record. Nitrogen and phosphorous increase in the Transition Zone and into D1 interpreted to be an effect of dam installation. See text for details.
Transition Zone—1888–1958 CE
The TZ is defined by an abrupt shift to a stairstep pattern in the CONISS between 36 and 31 cm (1888 and 1958 CE), which spans the period when Jackson Lake Dam was installed (1906–1916 CE; Figure 4). A shift in the abundances of Lindavia and Stephanodiscus occurs in the TZ (Figure 4). In general, L. ocellata declines in TZ with its last occurrence at 25 cm (1958 CE) (Figure 4). In contrast, the S. parvus-minutulus complex increases in abundance during the TZ, averaging ∼30% of the total assemblage with highest peaks reaching ∼50%. The only occurrence of S. niagarae above 5% occurs in the middle of the TZ, corresponding to a large peak in P (Figures 4, 5). Diatom concentrations show a slight increasing trend throughout the TZ (Figure 5). The L:S ratio shows an abrupt shift from positive to negative values at the onset of TZ (Figure 4). The shift to negative L:S values remain consistent throughout the interval. Ratios of Si:Ti are highly variable over the TZ with no observable trend (Figure 5). Total nitrogen concentrations exhibit a variable positive trend ranging from ∼0.2 to ∼0.3 wt. % (Figure 5). Phosphorous concentrations show a highly variable positive trend throughout the TZ. The largest peak in P occurs between 1938 and 1943 CE (Figure 5).
Diatom Zone 2 1958–2019 CE
Diatom Zone 2 is characterized by Stephanodiscus-dominated plankton (Figure 4). Lindavia intermedia first appears >5% in 1980 and persists until 2007 CE (Figure 4). Stephanodiscus parvus-minutulus complex increases in abundance across D2, reaching 50%–60% from 1958 to 1971 CE and 1987–2019 CE (Figure 4). Abundances of A. formosa are variable between 1888 and 1978 CE with less variability between 1983 and 2007 CE. Fragilaria crotonensis is observed from 1978 to 2002 CE with abundances of ∼5–25% but is largely absent elsewhere in D2. Diatom concentrations maintain a positive trend throughout D2 (Figure 5). The L:S ratio is consistently negative in D2 (Figure 4). From 1946 to 1958 CE, L:S is variable, like the TZ. After 1958 CE, L:S largely maintains a value of −1. The Si:Ti shows a weakly positive trend in D2 (Figure 5). Total nitrogen values range from ∼0.1 to 0.4 wt. %. Phosphorus counts remain highly variable with higher overall values than D1.
Discussion
Overview
In aquatic ecosystems such as Jackson Lake, the absence of long monitoring records requires paleolimnological archives be used for retrospective environmental assessment and establishing baselines for change detection (Smol, 2019). Diatom-rich lake sediments have proven valuable for evaluating both short and long-term natural and anthropogenic changes to ecosystem structure and function worldwide (e.g., Dixit et al., 1993; Flower et al., 2001; Haberle et al., 2006; Woodbridge et al., 2014; Chen et al., 2015; Maslennikova et al., 2016; Smol, 2019; Halac et al., 2020; Streib et al., 2021). Such information provides valuable context for lake managers seeking to preserve ecosystem services as hydroclimatic conditions change (Paterson et al., 2020). When considering Jackson Lake and dam construction, knowledge of the impacts to primary productivity and algal assemblages are critical; phytoplankton form the base of the aquatic food web and are responsible for transferring energy to higher trophic levels. Further, algal production impacts water clarity and chemistry, important variables for lakes that provide cultural services (Goldman, 1988; Berman et al., 1995; Wang et al., 2016; Jia et al., 2019).
For Jackson Lake, Stephanodiscus and Lindavia are valuable for understanding limnological changes associated with hydroclimate and dam installation, especially considering the deep-water location of core 9B. In addition, A. formosa and F. crotonensis provide insights on TN concentrations, with F. crotonensis favoring higher Si:N ratios than A. formosa (Saros et al., 2011; Slemmons et al., 2017). Heavily silicified diatoms like A. subarctica favor higher Si:P conditions (Kilham et al., 1996). At higher P concentrations, populations disappear rapidly, due to competition from centric diatoms with higher nutrient thresholds (Anderson et al., 1997; Gibson et al., 2003). In addition, abundances of Aulacoseira spp. can indicate periods of vertical mixing and more turbid waters (Reynolds et al., 2002; Garzon and Saros, 2022). Likewise, F. crotonensis abundances can be interpreted to indicate well-mixed water columns or turbid conditions (Bailey-Watts, 1986).
Diatom Zone 1—1654–1888 CE
The diatoms of D1 reveal that nutrient deficient conditions were common in Jackson Lake prior to dam installation, and that regional climate during the Little Ice Age (LIA) may have played an important role in lake function during this period. The pervasiveness of L. ocellata throughout D1 indicates that Jackson Lake had low concentrations of N and P, indicating oligotrophic conditions over most of this period (Winder et al., 2008; Saros and Anderson, 2015; Streib et al., 2021). Low nutrient concentrations limited blooms of diatoms dependent on higher nutrient thresholds, even during transient periods of mixing. Supporting this interpretation is the L:S ratio, which shows dominantly positive values throughout D1. Similarly, the chemostratigraphic data show low values and high variability of P in D1. We interpret this signal as consistent with the nutrient limitations that favored Lindavia over Stephanodiscus (Streib et al., 2021). The relative abundances of Aulacoseira and F. crotonensis within D1 suggests that Si was periodically abundant, perhaps associated with occasional turbulent mixing (Bailey-Watts, 1986; Bormans and Webster, 1999; Reynolds et al., 2002; Garzon and Saros, 2022; Diatoms of North America). Increases in F. crotonensis often coincide with declines in A. formosa in D1 (Figure 4). The higher relative Si/P is favorable for F. crotonensis. Both A. formosa and F. crotonensis have similar nutrient demands, with A. formosa being the better competitor in N-limited systems (Saros et al., 2005). Intervals characterized by relatively abundant A. formosa are interpreted to reflect N-limitations in Jackson Lake, while periods of more abundant N and Si favor F. crotonensis (Bennion et al., 2011).
Diatom Zone 1 encompasses the end of the LIA, which had profound effects on the regional climate. Tree-ring evidence collected from the Greater Yellowstone Ecosystem (GYE) shows that the climate of the middle to late LIA (1600–1850 CE) was marked by long cold winters and short cool summers (Rochner et al., 2021). Lake stratification appears to have been influenced by these conditions. Jacobs and Whitlock (2008) used paleo-vegetation data to suggest that the LIA was considerably wetter than earlier periods in the GYE. Two departures from the trend of cold wet winters are suggested by our data, first at ∼1710 CE and again at 1726 CE. During those years, increases in Stephanodiscus (lower L:S) are interpreted to indicate greater availability of nutrients in Jackson Lake, likely driven by more complete spring mixing. A similar interpretation for Crevice Lake (Montana, USA) was made by Whitlock et al. (2008) using multi-proxy lake records. From 1700 to 1800 CE, Crevice Lake was interpreted to have more complete spring turnover, which was attributed to milder winters and warmer summers (Whitlock et al., 2008).
Transition Zone—1888–1958 CE
Prior to start of the TZ, data suggests oligotrophic conditions with planktic assemblages dominated by Lindavia. At onset of the TZ, L:S ratios shifted to negative values, indicating greater nutrient availability in the photic zone that spurred a change in diatom composition. This assemblage change occurred rapidly, over about a decade, as indicated by the hierarchical change in the CONISS (Figure 4). Rapid successions in diatom assemblages and abundance changes have occurred in other aquatic systems modified by dams, for example at Lake Ford (Michigan, USA) and on the Liuxihe Reservoir in southern China (Donar et al., 1996; Liu et al., 2012). In the case of Jackson Lake, the timeline of changes in the diatoms suggests that climate change at the end of the LIA drove succession in the mid-19th century (Whitlock et al., 2008; Brown et al., 2021), followed and amplified by the effects of dam installation thereafter. With warming following the LIA, spring water column turnover may have started earlier, driving nutrient cycling and leading to blooms of Stephanodiscus, the dominant diatoms throughout the TZ. This interpretation is supported by highly variable abundances of Aulacoseira, which was in decline as Stephanodiscus utilized available P. Similarly, TN and P both increase during the TZ (Figure 5). The Stephanodiscus parvus-minutulus complex saw the largest increase, with Stephanodiscus niagarae only reaching abundances >5% in one instance. Interestingly, abundant S. niagarae correlates with the largest peak in P in the core record (Figure 5). The effects of dam emplacement on nutrient supply and cycling dynamics in new reservoirs has been studied elsewhere, but data for Jackson Lake were unavailable prior to our study (e.g., Medeiros et al., 2011; Maavara et al., 2020; Yan et al., 2021). The Jackson Lake Dam’s flooding of supralittoral wetlands very likely resulted in a rapid introduction of nutrients into the reservoir, driving the decline of L. ocellata (Figure 4) (Baxter, 1977). A trend of increasing nutrient abundance crossing into the post-dam period is corroborated in the geochemical data (Figure 5).
Climatic conditions during the period encompassed by the TZ (1888–1958 CE) varied, with frequent droughts, higher than average temperatures, and variability in Snake River discharge (Figure 2). During the Dust Bowl (1930s CE), PDSI data show western Wyoming experienced several years of sustained drought (NOAA, 2021a; NOAA, 2021b). These conditions are reflected in reduced Snake River discharge (Wise, 2010). Installation of the Jackson Lake Dam and frequent droughts contributed to frequent variations in lake water level from 2,051 to 2,062 masl (Figure 2). The long-term effects of droughts on diatom populations in the pelagic environment were minimal. Diatoms can recover from droughts and establish mature communities in 2–4 weeks (Oemke and Burton, 1986; Calapez et al., 2014). The high temporal resolution of our diatom record allows us to examine the impacts of the 1930s droughts on Jackson Lake’s diatoms with minimal time averaging. In the TZ, Stephanodiscus remains as the dominate species despite the droughts, but this may relate to the deep-water offshore environment of our sediment record. This setting may have been minimally affected by small changes in water level in comparison to shallower, nearshore environments. We therefore interpret that the major factor controlling Stephanodiscus in the TZ was nutrient abundance and availability associated with dam installation, with secondary influences of climate.
Mean monthly water-levels after dam installation show frequent transgressions and regressions of 5–9 m (Figure 2). This coincides with increased variability in the negative L:S values (Figure 5). These trends of lake-level and L:S variability continue from establishment of the dam until 1958 CE. Stephanodiscus varied in abundance (25%–50%) between 1943 and 1958 CE (Figure 4). During this period, average monthly water levels were highly variable, ranging between 2,052 and 2,063 masl. The 5–9 m reductions in water level seem to correlate with declines in Stephanodiscus abundances and may be a contributing factor in their declines (Figures 2, 5). A possible explanation for the relationship is that lower water-levels coincide with reduced lake surface area, limiting the habitat available for planktic diatoms. Water level changes between 2,051 and 2,062 masl exposed and reflooded peripheral wetlands, which would have mobilized nutrients from the wetlands into the water column. This can partially explain increases in TN and P during this period (Figure 5). The L:S ratio from the end of the TZ through D2 is negative, showing a variable trend from 1943 to 1958 CE, but generally signifying relatively abundant nutrients compared to D1. Ultimately, establishment of the dam and the transition of Jackson Lake to a reservoir resulted in a departure from the nutrient status inferred for D1 into a transitional phase represented by TZ (Figure 5). Driving this departure is a combination of likely increased residence times and nutrient availability resulting from dam emplacement and warming temperatures, which are reflected in an increase in Stephanodiscus and a decline in Lindavia, as well as heavily silicified diatom species.
Diatom Zone 2 1958–2019 CE
Zone D2 is characterized by abundant Stephanodiscus and the absence of L. ocellata. Small Stephanodiscus species experienced their largest assemblage increases, reaching as high as 70% of the total (Figure 4). We interpret the dominance of Stephanodiscus in D2 to reflect an increase in nutrient availability, particularly an abundance of P in the photic zone (Reavie et al., 1995). The L:S ratio from 1958 to 2019 CE is very different from the trends observed in D1 and TZ. From 1958 to 2019 CE, the L:S ratio becomes strongly negative, departing from the maximum value of −1 in three instances. Abundant Stephanodiscus species and negative L:S values suggest a more mesotrophic lake state, an interpretation consistent with the relationship inferred for these diatoms and nutrient status in other aquatic systems (Bradbury, 1988; Kilham et al., 1996; Bracht et al., 2008).
The sharp decline in Stephanodiscus abundance at ∼1986 CE is interpreted to be linked to a reduction in TN and P concentrations and the sediment signal produced by a turbidite (McGlue et al., 2023) (Figure 5). The reduction in nutrient concentrations and decline in Stephanodiscus abundances correspond to an increase in Aulacoseira spp., Asterionella spp. and Fragilaria crotonensis, which were more efficient at competing for limited P (Figure 4). Major declines in Stephanodiscus during D2 coincide with declines in water level, suggesting a sensitivity to hydroclimate. The sharp declines in reservoir level may be a combined result of modulated reservoir outflow with increased evaporation during drought conditions.
Lindavia ocellata does not appear in abundances >5% in D2. The sharp decline in L. ocellata occurs in tandem with a sharp increase in Stephanodiscus spp. We interpret that this transition resulted from nutrient loading following dam installation, repeated transgressions from reservoir water level changes (as plants decayed and soil nutrients were mobilized), and more efficient internal cycling (mixing) at seasonal transitions (e.g., Reavie et al., 1995; Streib et al., 2021). The appearances of Lindavia intermedia in 1982, 1994, and 2007 CE as transient peaks generally correspond in time with declines in Stephanodiscus abundances. These periods are likely indicative of enhanced stratification (e.g., Bayer et al., 2016; Streib et al., 2021). Both 1982 and 1994 CE had above average annual mean temperatures, and 1982, 1994, and 2007 CE were also drought years, which supports the potential for enhanced stratification during these years. The L:S response during these periods remain negative, while XRF-derived P data was elevated (Figure 5).
Asterionella formosa has a consistent presence in D2 and is associated with increased abundance of N (Arnett et al., 2012). In comparison to the LIA, where F. crotonensis co-occurs with A. formosa, in D2 this species only occurs between ∼1977 and 2001 CE. The absences of F. crotonensis elsewhere in D2 indicates periods where N was the limiting nutrient (Arnett et al., 2012). Both A. formosa and F. crotonensis respond readily to increases in N (Michel et al., 2006). The abundances of A. formosa and F. crotonensis within the ∼1977 to 2001 CE period partially coincide with elevated values of N and Si (McGlue et al., 2023). Increases in A. formosa and F. crotonensis during this interval are attributed to increases in Si and N (Saros et al., 2005; Bennion et al., 2011). It is unlikely given the abundances of A. formosa and F. crotonensis that N was a limiting nutrient within the epilimnion during this period. Asterionella formosa and F. crotonensis largely replaced Aulacoseira and Lindavia during D2, showing a succession to species better able to compete under greater nutrient availability.
Geochemical data from core 9B show an overall increase in N and P concentrations moving towards the present, whereas Si and Ti show long-term declines; higher N and P concentrations appeared post dam emplacement and continued though the end of D2 (Figure 5). Diatom concentrations show an increase from D1 through D2, demonstrating that diatom productivity throughout the core record increased towards the present day. We interpret these data to indicate that trophic status shifted slightly towards a more mesotrophic state since dam completion and the corresponding transgression took place. The period after 1958 CE marks a new equilibrium state for Jackson Lake (Figure 5). The second equilibrium state of Jackson Lake is interpreted as being seasonally well-mixed, facilitating nutrient cycling, with more productive surface waters relative to D1. The new equilibrium diatom assemblage is characterized by large Stephanodiscus blooms replacing Aulacoseira following lake mixing, and replacement of Lindavia species with Asterionella and Fragilaria.
Rühland et al.’s (2008) evaluation of <200 non-acidified/non-enriched lakes in North America showed significant increases in abundances of planktic cyclotelloid species, with declines in heavily silicified Aulacoseira and Fragilaria taxa since the end of the LIA. This trend appears to be consistent with what is observed in our study following the end of the LIA ∼1850 CE (Figure 4). Both TZ and D2 show an increase in the relative abundances of small Stephanodiscus species and a decline in abundances of more heavily silicified species like Aulacoseira and Fragilaria (Figure 4). Similarly, Rühland et al. (2015) illustrated the success of smaller cyclotelloid species as anthropogenic climate warming began to change lake conditions (decrease in ice/snow cover, strength of thermal stratification, etc.).
Conclusion
This study has developed the first sediment core-based diatom record for Jackson Lake from 1654 to 2019 CE and provides a new baseline for interpreting environmental and anthropogenic changes to this aquatic ecosystem. The record shows that prior to the 19th century, Jackson Lake’s plankton were dominated by Lindavia. The diatoms responded to climate changes at the termination of the LIA by the mid-19th century, in evidence from greater abundances of Stephanodiscus from ∼1850 to 1907 CE, which likely tracked a longer ice-free period and improved internal nutrient cycling. This implies that natural climate changes impart a strong control on limnological processes and algal composition in Jackson Lake.
Construction of the Jackson Lake Dam resulted in substantial changes to the lake surface elevation, morphology, and discharge at the outlet. After dam emplacement, a transition in the diatom assemblage occurred rapidly, with Stephanodiscus replacing Lindavia as the dominate planktic species within a decade. This transition implies (1) an overall increase in nutrient concentrations and perhaps more efficient seasonal nutrient cycling and (2) a shift in trophic state from oligotrophic to more mesotrophic as primary productivity increased. Despite this transition, evidence for eutrophication is absent, suggesting management of the water body has not compromised environmental integrity. The outcomes of this study provide new perspective on the impacts of human water body engineering and climate change that will be valuable for conserving Jackson Lake’s ecosystem services in the future.
Data Availability Statement
The raw data supporting the conclusion of this article will be made available by the authors, without undue reservation.
Author Contributions
Project conceptualization—MM, JD, and JS. Original draft, formal analysis, and data visualization—JD. Project supervision—JS, MM, KY, and RT. Draft review and editing—All authors. Data resources, methodology, and laboratory facilities—JS, KY, and MM. Project funding and management—RT, KY, and MM.
Funding
The research was generously funded and supported by the U.S. National Science Foundation Tectonics program (Award #1932808), the Pioneer Endowment, and the Overcash Family Fund for Field Research at the University of Kentucky.
Conflict of Interest
The authors declare that the research was conducted in the absence of any commercial or financial relationships that could be construed as a potential conflict of interest.
Publisher’s Note
All claims expressed in this article are solely those of the authors and do not necessarily represent those of their affiliated organizations, or those of the publisher, the editors and the reviewers. Any product that may be evaluated in this article, or claim that may be made by its manufacturer, is not guaranteed or endorsed by the publisher.
Acknowledgments
We wish to thank the staff of Colter Bay and Signal Mountain marinas for their assistance and support of the field work. We also thank the Continental Scientific Drilling Facility for providing the services and facilities for core description, subsampling, and archival support for this project. We appreciate the constructive comments of our reviewers and Associate Editor Al Suwaidi for improvements to an earlier version of the manuscript.
Supplementary Material
The Supplementary Material for this article can be found online at: https://www.escubed.org/articles/10.3389/esss.2023.10065/full#supplementary-material
Supplementary Figure S1 | Scanning electron microscope (SEM) images of major diatom species found in core GTGC-J19-9B-1G-1. (A) Fragilaria crotonensis. (B) Lindavia ocellata. (C) Lindavia Intermedia. (D) Stephanodiscus niagarae. (E) Stephanodiscus rugosa. (F) Stephanodiscus cf medius. (G) Stephanodiscus hantzschii and an example of Stephanodiscus cf medius. (H) Shows additional examples of Stephanodiscus rugosa and Stephanodiscus hantzschii.
References
Anderson, N. J., Blomqvist, P., and Renberg, I. (1997). An Experimental and Palaeoecological Study of Algal Responses to Lake Acidification and Liming in Three Central Swedish Lakes. Eur. J. Phycol. 32, 35–48. doi:10.1080/09541449710001719355
Arnett, H. A., Saros, J. E., and Alisa Mast, M. (2012). A Caveat Regarding Diatom-Inferred Nitrogen Concentrations in Oligotrophic Lakes. J. Paleolimnol. 47, 277–291. doi:10.1007/s10933-011-9576-z
Bailey-Watts, A. E. (1986). The Ecology of Planktonic Diatoms, Especially Fragilaria Crotonensis, Associated with Artificial Mixing of a Small Scottish Loch in Summer. Diatom Res. 2, 153–168. doi:10.1080/0269249X.1986.9704966
Battarbee, R. W., Cameron, N. G., Golding, P., Brooks, S. J., Switsur, R., Harkness, D., et al. (2001a). Evidence for Holocene Climate Variability from the Sediments of a Scottish Remote Mountain Lake. J. Quat. Sci. Publ. Quat. Res. Assoc. 16, 339–346. doi:10.1002/jqs.597
Battarbee, R. W., Jones, V. J., and Flower, R. J. (2001b). “Diatoms,” in Tracking Environmental Change Using Lake Sediments. Terrestrial, Algal and Siliceous Indicators. Editors J. P. Smol, and H. J. B. Birks, Vol. 3, 155.
Battarbee, R. W., and Kneen, M. J. (1982). The Use of Electronically Counted Microspheres in Absolute Diatom Analysis. Limnol. Oceanogr. 27, 184–188. doi:10.4319/lo.1982.27.1.0184
Baxter, R. M. (1977). Environmental Effects of Dams and Impoundments. Annu. Rev. Ecol. Syst. 8, 255–283. doi:10.1146/annurev.es.08.110177.001351
Bayer, T. K., Schallenberg, M., and Burns, C. W. (2016). Contrasting Controls on Phytoplankton Dynamics in Two Large, Pre-alpine Lakes Imply Differential Responses to Climate Change. Hydrobiologia 771, 131–150. doi:10.1007/s10750-015-2625-2
Bennion, H., Simpson, G. L., John Anderson, N., Clarke, G., Dong, X., Hobæk, A., et al. (2011). Defining Ecological and Chemical Reference Conditions and Restoration Targets for Nine European Lakes. J. Paleolimnol. 45, 415–431. doi:10.1007/s10933-010-9418-4
Berman, T., Stone, L., Yacobi, Y. Z., Kaplan, B., Schlichter, A., Nishri, A., et al. (1995). Primary Production and Phytoplankton in Lake Kinneret: A Long-Term Record (1972-1993). Limnol. Oceanogr. 40, 1064–1076. doi:10.4319/lo.1995.40.6.1064
Bormans, M., and Webster, I. T. (1999). Modelling the Spatial and Temporal Variability of Diatoms in the River Murray. J. Plankton Res. 21, 581–598. doi:10.1093/plankt/21.3.581
Bracht, B. B., Stone, J. R., and Fritz, S. C. (2008). A Diatom Record of Late Holocene Climate Variation in the Northern Range of Yellowstone National Park, USA. Quat. Int. 188, 149–155. doi:10.1016/j.quaint.2007.08.043
Bradbury, J. (1988). A Climatic–Limnologic Model of Diatom Succession for Paleolimnological Interpretation of Varved Sediments in Elk Lake, Minnesota. J. Paleolimnol. 1, 115–131.
Brown, S. R., Cartier, R., Schiller, C. M., Zahajská, P., Fritz, S. C., Morgan, L. A., et al. (2021). Multi-proxy Record of Holocene Paleoenvironmental Conditions from Yellowstone Lake, Wyoming, USA. Quat. Sci. Rev. 274, 107275. doi:10.1016/j.quascirev.2021.107275
Byrd, J. O., Smith, R. B., and Geissman, J. W. (1994). The Teton Fault, Wyoming: Topographic Signature, Neotectonics, and Mechanisms of Deformation. J. Geophys. Res. Solid Earth 99, 20095–20122. doi:10.1029/94jb00281
Calapez, A. R., Elias, C. L., Almeida, S. F., and Feio, M. J. (2014). Extreme Drought Effects and Recovery Patterns in the Benthic Communities of Temperate Streams. Limnetica 33, 281–296. doi:10.23818/limn.33.22
Chen, G., Shi, H., Tao, J., Chen, L., Liu, Y., Lei, G., et al. (2015). Industrial Arsenic Contamination Causes Catastrophic Changes in Freshwater Ecosystems. Sci. Rep. 5, 17419–17427. doi:10.1038/srep17419
Clark, M. L., Sadler, W. J., and O’Ney, S. (2004). Water-Quality Characteristics of the Snake River and Five Tributaries in the Upper Snake River Basin, Grand Teton National Park, Wyoming, 1998–2002. Collingdale, PA, USA: DIANE Publishing.
Curtis, J., and Grimes, K. (2004). Wyoming Climate Atlas. Laramie, Wyoming: Office of the Wyoming State Climatologist, University of Wyoming. Available at: http://www.wrds.uwyo.edu/sco/climateatlas/(Accessed November, 2021).
Daugherty, J., Crockett, S., Goetzmann, W. H., and Jackson, R. G. (1999). A Place Called Jackson Hole: The Historic Resource Study of Grand Teton National Park. Moose, WY, USA: Grand Teton Association.
Dixit, S. S., Cumming, B. F., Birks, H. J. B., Smol, J. P., Kingston, J. C., Uutala, A. J., et al. (1993). Diatom Assemblages from Adirondack Lakes (New York, USA) and the Development of Inference Models for Retrospective Environmental Assessment. J. Paleolimnol. 8, 27–47. doi:10.1007/bf00210056
Donar, C. M., Neely, R. K., and Stoermer, E. F. (1996). Diatom Succession in an Urban Reservoir System. J. Paleolimnol. 15 (3), 237–243. doi:10.1007/bf00213043
Finger, D., Schmid, M., and Wueest, A. (2006). Effects of Upstream Hydropower Operation on Riverine Particle Transport and Turbidity in Downstream Lakes. Water Resour. Res. 42 (8). doi:10.1029/2005wr004751
Flower, R. J., Dobinson, S., Ramdani, M., Kraïem, M. M., Ben Hamza, C., Fathi, A. A., et al. (2001). Recent Environmental Change in North African Wetland Lakes: Diatom and Other Stratigraphic Evidence from Nine Sites in the CASSARINA Project. Aquat. Ecol. 35, 369–388. doi:10.1023/a:1011984627760
Garzon, E. L. P., and Saros, J. E. (2022). Ecology of the Diatom Aulacoseira Pusilla in Oligotrophic Mountain Lakes, with Implications for Paleoclimate Reconstructions. Arct. Antarct. Alp. Res. 54 (1), 147–162. doi:10.1080/15230430.2022.2071088
Gibson, C. E., Anderson, N. J., and Haworth, E. Y. (2003). Aulacoseira Subarctica: Taxonomy, Physiology, Ecology and Palaeoecology. Eur. J. Phycol. 38, 83–101. doi:10.1080/0967026031000094102
Goldman, C. R. (1988). Primary Productivity, Nutrients, and Transparency during the Early Onset of Eutrophication in Ultra-oligotrophic Lake Tahoe, Califomia-Nevada1. Limnol. Oceanogr. 33, 1321–1333. doi:10.4319/lo.1988.33.6.1321
Good, J. M., and Pierce, K. L. (1996). Interpreting the Landscapes of Grand Teton and Yellowstone National Parks: Recent and Ongoing Geology. Jackson Hole, Wyoming: Grant Teton Natural History Association.
Graf, W. L. (2001). Dam Age Control: Restoring the Physical Integrity of America’s Rivers. Ann. Assoc. Am. Geogr. 91, 1–27. doi:10.1111/0004-5608.00231
Graf, W. L. (2005). Geomorphology and American Dams: The Scientific, Social, and Economic Context. Geomorphology 71, 3–26. doi:10.1016/j.geomorph.2004.05.005
Guerreiro, R. L., McGlue, M. M., Stone, J. R., Bergier, I., Parolin, M., da Silva Caminha, S. A., et al. (2018). Paleoecology Explains Holocene Chemical Changes in Lakes of the Nhecolândia (Pantanal-Brazil). Hydrobiologia 815, 1–19.
Haberle, S. G., Tibby, J., Dimitriadis, S., and Heijnis, H. (2006). The Impact of European Occupation on Terrestrial and Aquatic Ecosystem Dynamics in an Australian Tropical Rain Forest. J. Ecol. 94, 987–1002. doi:10.1111/j.1365-2745.2006.01140.x
Halac, S., Mengo, L., Guerra, L., Lami, A., Musazzi, S., Loizeau, J. L., et al. (2020). Paleolimnological Reconstruction of the Centennial Eutrophication Processes in a Sub-tropical South American Reservoir. J. S. Am. Earth Sci. 103, 102707. doi:10.1016/j.jsames.2020.102707
Hostetler, S., Whitlock, C., Shuman, B., Liefert, D., Drimal, C. W., and Bischke, S. (2021). Greater Yellowstone Climate Assessment: Past, Present, and Future Climate Change in Greater Yellowstone Watersheds. Bozeman, MT, USA: Montana State University, Institute on Ecosystems.
Houk, V. (2010). Atlas of Freshwater Centric Diatoms with a Brief Key and Descriptions. Stephanodiscaceae A, Cyclotella, Tertiarius, Discostella. Fottea 10, 1–498.
Intralawan, A., Wood, D., Frankel, R., Costanza, R., and Kubiszewski, I. (2018). Tradeoff Analysis between Electricity Generation and Ecosystem Services in the Lower Mekong Basin. Ecosyst. Serv. 30, 27–35. doi:10.1016/j.ecoser.2018.01.007
Jacobs, K., and Whitlock, C. (2008). A 2000-year Environmental History of Jackson Hole, Wyoming, Inferred from Lake-Sediment Records. West. North Am. Nat. 68 (3), 350–364.
Jia, J., Gao, Y., Song, X., and Chen, S. (2019). Characteristics of Phytoplankton Community and Water Net Primary Productivity Response to the Nutrient Status of the Poyang Lake and Gan River, China. Ecohydrology 12, e2136. doi:10.1002/eco.2136
Johnson, S. E., Swallom, M., Thigpen, J. R., McGlue, M. M., Dortch, J., Gallen, S., et al. (2022). The Influence of Glacial Topography on Fluvial Efficiency in the Teton Range, Wyoming (USA). Earth Surf. Process. Landforms 592, 117643. doi:10.1016/j.epsl.2022.117643
Kamulali, T. M., McGlue, M. M., Stone, J. R., Kimirei, I. A., Goodman, P. J., and Cohen, A. S. (2022). Paleoecological Analysis of Holocene Sediment Cores from the Southern Basin of Lake Tanganyika: Implications for the Future of the Fishery in One of Africa’s Largest Lakes. J. Paleolimnol. 67, 17–34. doi:10.1007/s10933-021-00219-4
Kilham, S. S., Theriot, E. C., and Fritz, S. C. (1996). Linking Planktonic Diatoms and Climate Change in the Large Lakes of the Yellowstone Ecosystem Using Resource Theory. Limnol. Oceanogr. 41, 1052–1062. doi:10.4319/lo.1996.41.5.1052
Kylander, M., Ampel, L., Wohlfarth, B., and Veres, D. (2011). High-resolution X-Ray Fluorescence Core Scanning Analysis of Les Echets (France) Sedimentary Sequence: New Insights from Chemical Proxies. J. Quat. Sci. 26, 109–117. doi:10.1002/jqs.1438
Lehner, B., Liermann, C. R., Revenga, C., Vörösmarty, C., Fekete, B., Crouzet, P., et al. (2011). High-resolution Mapping of the World's Reservoirs and Dams for Sustainable River-Flow Management. Front. Ecol. Environ. 9, 494–502. doi:10.1890/100125
Liu, J., Lin, Z., Zhang, H., and Han, B. P. (2012). Hydrodynamic Change Recorded by Diatoms in Sediments of Liuxihe Reservoir, Southern China. Available at: https://pubag.nal.usda.gov/catalog/127186 (Accessed April, 2022).
Love, J. D., Reed, J. C., and Christiansen, A. C. (1992). Geologic Map of Grand Teton National Park, Teton County, Wyoming: U.S. Geol. Surv. IMAP USGS Numbered Ser. 2031. doi:10.3133/i2031
Love, J. D., and Reed, J. R. (1968). Creation of the Teton Landscape-The Geologic Story of Grand Teton National Park. Jackson Hole, Wyoming: Grand Teton Natural History Association.
Maavara, T., Chen, Q., Van Meter, K., Brown, L. E., Zhang, J., Ni, J., et al. (2020). River Dam Impacts on Biogeochemical Cycling. Nat. Rev. Earth Environ. 1, 103–116. doi:10.1038/s43017-019-0019-0
Maslennikova, A. V., Udachin, V. N., and Aminov, P. G. (2016). Lateglacial and Holocene Environmental Changes in the Southern Urals Reflected in Palynological, Geochemical and Diatom Records from the Lake Syrytkul Sediments. Quat. Int. 420, 65–75. doi:10.1016/j.quaint.2015.08.062
Matzinger, A., Schmid, M., Veljanoska-Sarafiloska, E., Patceva, S., Guseska, D., Wagner, B., et al. (2007). Eutrophication of Ancient Lake Ohrid: Global Warming Amplifies Detrimental Effects of Increased Nutrient Inputs. Limnol. Oceanogr. 52, 338–353. doi:10.4319/lo.2007.52.1.0338
McCartney, M. (2009). Living with Dams: Managing the Environmental Impacts. Water Policy 11, 121–139. doi:10.2166/wp.2009.108
McGlue, M. M., Dilworth, J. R., Johnson, H. L., Whitehead, S. J., Thigpen, J. R., Yeager, K. M., et al. (2023). Effect of Dam Emplacement and Water Level Changes on Sublacustrine Geomorphology and Recent Sedimentation in Jackson Lake, Grand Teton National Park (Wyoming, United States). Earth Sci. Syst. Soc. 3, 10066. doi:10.3389/esss.2023.10066
McGreevy, L. J., and Gordon, E. D. (1964). Ground Water East of Jackson Lake, Grand Teton National Park, Wyoming. 494. Reston, Virginia: US Department of the Interior, Geological Survey.
Medeiros, P. R. P., Knoppers, B. A., Cavalcante, G. H., and Souza, W. F. L. (2011). Changes in Nutrient Loads (N, P and Si) in the São Francisco Estuary after the Construction of Dams. Braz. Archives Biol. Technol. 54, 387–397. doi:10.1590/S1516-89132011000200022
Michel, T. J., Saros, J. E., Interlandi, S. J., and Wolfe, A. P. (2006). Resource Requirements of Four Freshwater Diatom Taxa Determined by In Situ Growth Bioassays Using Natural Populations from Alpine Lakes. Hydrobiologia 568, 235–243. doi:10.1007/s10750-006-0109-0
NOAA National Centers for Environmental information (2021a). Climate at a Glance: Statewide Time Series. Available at: https://www.ncdc.noaa.gov/cag/(Accessed October 21, 2021).
NOAA National Centers for Environmental information (2021b). Climate Data Online: Station Details. Available at: https://www.ncdc.noaa.gov/cdo-web/datasets/GHCND/stations/GHCND:USC00486440/detail (Accessed October 21, 2021).
Oemke, M. P., and Burton, T. M. (1986). Diatom Colonization Dynamics in a Lotic System. Hydrobiologia 139, 153–166. doi:10.1007/BF00028099
Paterson, A. M., Köster, D., Reavie, E. D., and Whitmore, T. J. (2020). Preface: Paleolimnology and Lake Management. Lake Reserv. Manag. 36, 205–209. doi:10.1080/10402381.2020.1805998
Pierce, K. L., and Haller, K. M. (2011). Fault Number 768b, Teton Fault, Northern Section, in Quaternary Fault and Fold Database of the United States. U.S. Geological Survey website. Available at: https://earthquakes.usgs.gov/hazards/qfaults.
Pierce, K. L., Licciardi, J. M., Good, J. M., and Jaworowski, C. (2018). Pleistocene Glaciation of the Jackson Hole Area, Wyoming. U.S. Geological Survey Professional Paper USGS Numbered Series 1835, 68. doi:10.3133/pp1835
Postel, S., and Carpenter, S. (1997). “Freshwater Ecosystem Services,” in Nature’s Services. Societal Dependence on Natural Ecosystems. Editor G. Daily (Washington DC: Island Press), 195.
Reavie, E. D., Hall, R. I., and Smol, J. P. (1995). An Expanded Weighted-Averaging Model for Inferring Past Total Phosphorus Concentrations from Diatom Assemblages in Eutrophic British Columbia (Canada) Lakes. J. Paleolimnol. 14, 49–67. doi:10.1007/BF00682593
Reynolds, C. S., Huszar, V., Kruk, C., Naselli-Flores, L., and Melo, S. (2002). Towards a Functional Classification of the Freshwater Phytoplankton. J. Plankton Res. 24 (5), 417–428. doi:10.1093/plankt/24.5.417
Rochner, M. L., Heeter, K. J., Harley, G. L., Bekker, M. F., and Horn, S. P. (2021). Climate-induced Treeline Mortality during the Termination of the Little Ice Age in the Greater Yellowstone Ecoregion, USA. Holocene 31, 1288–1303. doi:10.1177/09596836211011656
Rühland, K., Paterson, A. M., and Smol, J. P. (2008). Hemispheric-scale Patterns of Climate-Related Shifts in Planktonic Diatoms from North American and European Lakes. Glob. Change Biol. 14, 2740. doi:10.1111/j.1365-2486.2008.01670.x
Rühland, K., Paterson, A. M., and Smol, J. P. (2015). Lake Diatom Responses to Warming: Reviewing the Evidence. J. Paleolimnol. 54, 1–35. doi:10.1007/s10933-015-9837-3
Saros, J. E., and Anderson, N. J. (2015). The Ecology of the Planktonic Diatom Cyclotella and its Implications for Global Environmental Change Studies. Biol. Rev. 90, 522–541. doi:10.1111/brv.12120
Saros, J. E., Clow, D. W., Blett, T., and Wolfe, A. P. (2011). Critical Nitrogen Deposition Loads in High-Elevation Lakes of the Western US Inferred from Paleolimnological Records: Water, Air. Soil Pollut. 216, 193–202. doi:10.1007/s11270-010-0526-6
Saros, J. E., Michel, T. J., Interlandi, S. J., and Wolfe, A. P. (2005). Resource Requirements of Asterionella Formosa and Fragilaria Crotonensis in Oligotrophic Alpine Lakes: Implications for Recent Phytoplankton Community Reorganizations. Can. J. Fish. Aquatic Sci. 62, 1681–1689. doi:10.1139/f05-077
Sawakuchi, A. O., Hartmann, G. A., Sawakuchi, H. O., Pupim, F. D. N., Bertassoli, D. J., Parra, M., et al. (2015). The Volta Grande Do Xingu: Reconstruction of Past Environments and Forecasting of Future Scenarios of a Unique Amazonian Fluvial Landscape. Sci. Drill. 20, 21–32. doi:10.5194/sd-20-21-2015
Slemmons, K. E. H., Rodgers, M. L., Stone, J. R., and Saros, J. E. (2017). Nitrogen Subsidies in Glacial Meltwaters Have Altered Planktonic Diatom Communities in Lakes of the US Rocky Mountains for at Least a Century. Hydrobiologia 800, 129–144. doi:10.1007/s10750-017-3187-2
Smith, R. B., Pierce, K. L., and Wold, R. J. (1993). “Seismic Surveys and Quatemary History of Jackson Lake, Wyoming,” in Geology of Wyoming, Mere. 5. Editors A. Snoke, J. R. Steitdmann, and S. Roberts (Laramie: Geologic Survey of Wyoming), 668–693.
Smol, J. P., and Stoermer, E. F. (2010). The Diatoms: Applications for the Environmental and Earth Sciences. 2nd ed. Cambridge, UK: Cambridge University Press.
Smol, J. P. (2019). Under the Radar: Long-Term Perspectives on Ecological Changes in Lakes. Proc. R. Soc. B Biol. Sci. 286, 20190834. doi:10.1098/rspb.2019.0834
Song, C., Omalley, A., Roy, S. G., Barber, B. L., Zydlewski, J., and Mo, W. (2019). Managing Dams for Energy and Fish Tradeoffs: What Does a Win-Win Solution Take? Sci. Total Environ. 669, 833–843. doi:10.1016/j.scitotenv.2019.03.042
Spaulding, S. A., Lubinski, D. J., and Potapova, M. (2010). Diatoms of the United States. Available at: http://westerndiatoms.colorado.edu.
Stene, E. A. (1997). The Minidoka Project. Washington, DC, USA: Bureau of Reclamation History Program, 102.
Stone, J. R., Edlund, M. B., Streib, L., Ha Quang, H., and McGlue, M. M. (2020). Stephanodiscus Coruscus Sp. nov., a New Species of Diatom (Bacillariophyta) from June Lake, California (USA) with Close Affiliation to Stephanodiscus Klamathensis. Diatom Res. 35, 339–351. doi:10.1080/0269249X.2020.1846079
Streib, L., Stone, J., Lyon, E., Ha, H., Yeager, K., Zimmerman, S., et al. (2021). Anthropogenic Climate Change Has Altered Lake State in the Sierra Nevada (California, USA). Glob. Change Biol. 27, 6059. doi:10.1111/gcb.15843
Thigpen, R., Brown, S. J., Helfrich, A. L., Hoar, R., McGlue, M., Woolery, E., et al. (2021). Removal of the Northern Paleo-Teton Range along the Yellowstone Hotspot Track. Lithosphere 2021, 1–27. doi:10.2113/2021/1052819
Thomas, C. C., and Koontz, L. (2021). 2020 National Park Visitor Spending Effects: Economic Contributions to Local Communities, States, and the Nation. Natural Resource Report NPS/NRSS/EQD/NRR—2021/2259. Fort Collins, Colorado: National Park Service. doi:10.36967/nrr-2286547
United States Geological Survey (1978). Geological Survey Research 1978, 464. U.S. Geological Survey, Professional Paper series 1100. doi:10.3133/pp1100
U.S. Bureau of ReclamationProject HistoryMinidoka Project (1928). Reclamation, Project History, 1929, 62; Reclamation, Project History, Minidoka Project, 1948, 20; Water and Power Resources, Project Data. 646. John Dooley, 6 June 1995.U.S. Bureau of Reclamation, Annual Project History, Minidoka Project (1912). Record Group 115, 3. Peterson, Idaho. 124, 44-45. Hereafter Record Group 115 Cited as RG 115.
Wang, J., Jiang, X., Zheng, B., Chen, C., Kang, X., Zhang, C., et al. (2016). Effect of Algal Bloom on Phosphorus Exchange at the Sediment–Water Interface in MeiliangBay of Taihu Lake, China. Environ. Earth Sci. 75, 57–59. doi:10.1007/s12665-015-4810-z
Whitlock, C., Dean, W., Rosenbaum, J., Stevens, L., Fritz, S., Bracht, B., et al. (2008). A 2650- Year-Long Record of Environmental Change from Northern Yellowstone National Park Based on a Comparison of Multiple Proxy Data. Quat. Int. 188, 126–138. doi:10.1016/j.quaint.2007.06.005
Wild, T. B., Reed, P. M., Loucks, D. P., Mallen-Cooper, M., and Jensen, E. D. (2019). Balancing Hydropower Development and Ecological Impacts in the Mekong: Tradeoffs for Sambor Mega Dam. J. Water Resour. Plan. Manag. 2, 05018019. doi:10.1061/(asce)wr.1943-5452.0001036
Winder, M., Reuter, J., and Schladow, S. (2008). Lake Warming Favours Small-Sized Planktonic Diatom Species. Proc. R. Soc. B Biol. Sci. 276, 427–435. doi:10.1098/rspb.2008.1200
Wise, E. (2010). Tree Ring Record of Streamflow and Drought in the Upper Snake River. Water Resour. Res. 46. doi:10.1029/2010WR009282
Woodbridge, J., Davies, H. J., Blake, W. H., and Fyfe, R. M. (2014). Recent Environmental Change in an Upland Reservoir Catchment: a Palaeoecological Perspective. J. Paleolimnol. 52, 229–244. doi:10.1007/s10933-014-9790-6
World Commission on Dams (WCD) (2000). Dams and Development: A New Framework for Decision-Making: The Report of the World Commission on Dams. London: Earthscan.
Wurtsbaugh, W. (2014). Jackson Lake Limnology. Logan, Utah: Watershed Sciences Faculty Publications, 559. Available at: https://digitalcommons.usu.edu/wats_facpub/559.
Wyoming Game and Fish Department (2021). Wyoming Game and Fish Department Fish Stocking Report. Available at: https://wgfapps.wyo.gov/FishStock/FishStock.
Yan, X., Thieu, V., and Garnier, J. (2021). Long-term Assessment of Nutrient Budgets for the Four Reservoirs of the Seine Basin (France). Sci. Total Environ. 778, 146412. doi:10.1016/j.scitotenv.2021.146412
Keywords: Paleoecology, diatoms, Jackson Lake, lake sediments, reservoir
Citation: Dilworth J, Stone JR, Yeager KM, Thigpen JR and McGlue MM (2023) Fossil Diatoms Reveal Natural and Anthropogenic History of Jackson Lake (Wyoming, USA). Earth Sci. Syst. Soc. 3:10065. doi: 10.3389/esss.2023.10065
Received: 17 July 2022; Accepted: 10 February 2023;
Published: 27 February 2023.
Edited by:
Aisha Al Suwaidi, Khalifa University, United Arab EmiratesReviewed by:
Andrew Medeiros, Dalhousie University, CanadaDenise Bicudo, Instituto de Pesquisas Ambientais (IPA), Brazil
Agnieszka Gałuszka, Jan Kochanowski University, Poland
Copyright © 2023 Dilworth, Stone, Yeager, Thigpen and McGlue. This is an open-access article distributed under the terms of the Creative Commons Attribution License (CC BY). The use, distribution or reproduction in other forums is permitted, provided the original author(s) and the copyright owner(s) are credited and that the original publication in this journal is cited, in accordance with accepted academic practice. No use, distribution or reproduction is permitted which does not comply with these terms.
*Correspondence: John Dilworth, john.dilworth@uky.edu